Leveraging Nature’s Self-Assembling Abilities to Engineer New Geometries
Leveraging Nature’s Self-Assembling Abilities to Engineer New Geometries
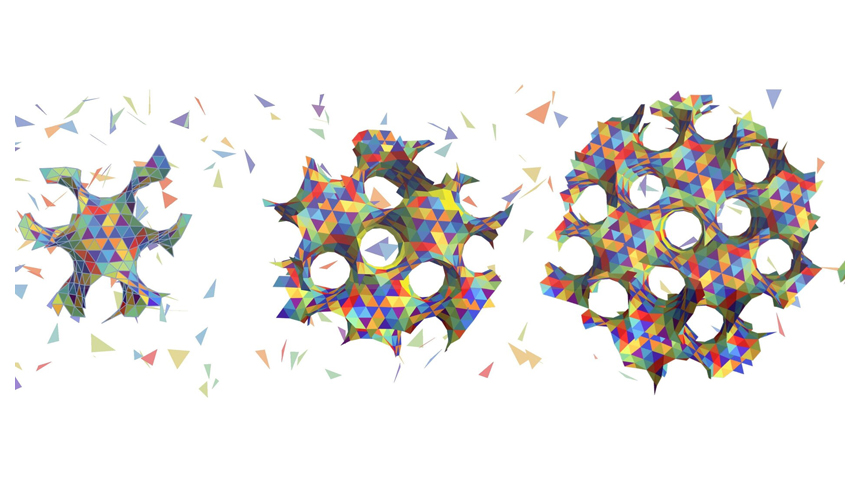
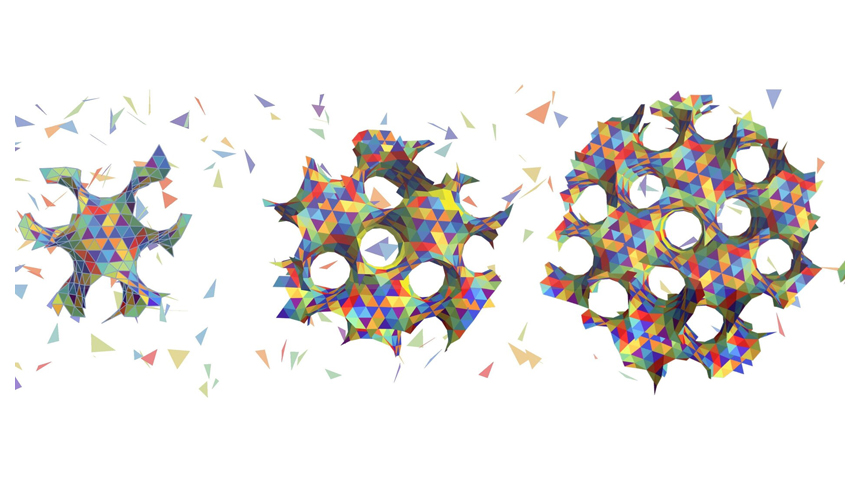
A multi-institutional team of researchers has developed a new computational model that supports maximum design economy for nano-sized self-assembling protein materials.
Viruses are a prime example of an organism that has perfected biological economy.
These small infectious agents are made up of a single molecule of nucleic acid, surrounded by a protein coat known as the capsid. This rigid, highly symmetric shell is built with the fewest number of proteins possible, said Greg Grason, a professor of polymer science at the University of Massachusetts Amherst. By exercising such conservation in its shell’s self-assembly, the virus is small enough to infect the cell, but not so small that it lacks the power to hijack the cell’s internal machinery and take over.
“Self-assembled materials in biological systems, or the structures that form inside of cells and tissues, are what give them their functional properties,” Grason said. “They usually build them out of protein building blocks. Some examples of these self-assembling materials are containers or shells that capture things or transport things from here to there. They also make a range of mechanical materials.”
Mother Nature has adapted these self-assembling proteins to determine function largely by controlling the size of the resulting structure. Scale is a key element of control that allows you to take the same building blocks and get different types of material functions, Grason said.
Discover the Benefits of ASME Membership
After colleagues at Brandeis University and the Technical University of Munich were able to leverage protein self-assembly to create antibody-coated shells to trap viruses, Grason and his research team wondered if they could do the same with a different geometry.
“They used spherical shells. We were interested in whether or not you could take the same kind of design principles and kind of flip it inside out and go from a positive curvature to one that is curved like a saddle,” he explained. “That kind of structure can give you a foamy, periodic, essentially crystalline arrangements. And it turns out that biology makes very similar architectures in bird feathers, beetles, and butterfly wings that gives them structural color.”
To do so, Grason and colleagues at Brandeis University and Syracuse University developed a computational model, harnessing Caspar-Klug symmetry principles. These principles, uncovered by biologists Donald Caspar and Nobel Prize winner Aaron Klug, govern how to build a structure with the largest possible volume with the fewest number of biological building blocks.
“We’ve been bootstrapping on some advances in the field of DNA nanotechnology, which hijacks DNA to make structural elements and, these days, making them into programmable, self-assembling building blocks, or subunits, that are about an order of magnitude larger than a protein but still have many of the same features of a protein,” he said. “So, we created a model to understand if there was a certain optimally economical way to program these subunits to create this particular geometry.”
Editor's Choice: Mapping 2D Ferroelectric Materials Unveils Connections
The group learned that having minimal surface property is essential to having extra symmetry. In other words, the inside of the surface is the same as the outside, so you can flip the subunit inside out to save on the number of subunits needed by a factor of two.
“The key was trying to figure out the connection between the symmetry of the structure and the economy of what you would need to achieve that structure,” said Grason.
As for next steps, the group is now working to realize the model experimentally, Grason said. Achieving economy is not the only challenge—now the team must work on how the different subunits bind together. Being able to repurpose symmetry principles to achieve structure control from the bottom-up self-assembly is a key advance; however, by introducing economy, you can also introduce possibilities for new defects that must be optimized.
“We are seeing that you have to be much more careful with the engineering and controlling when these subunits bind together, how tolerant they are to even very small deformations in the angles where they come together,” Grason said. “It’s not just about knowing which lock fits into which key, but there also needs to be a certain precision of where the angles are. It’s forcing us to go back to our collaborators and ask them to re-engineer their building blocks so they can come together.”
Eventually, it may be possible for engineers to “functionalize” this material for different applications, he continued.
“You could inject it or coat it on to some surface and have it self-assemble into a photonic coating,” Grason said. “That would be the next step to make it into a functional material for an application. It’s still one or two steps away, but is not actually quite as far away from reality as it might seem.”
Kayt Sukel is a technology writer and author in Houston.